Of the four fundamental forces – electromagnetic, weak, strong, and gravitational – gravity is by far the weakest. Yet at large distances it dominates all other interactions, governing the motion of astrophysical bodies from the planetary to the cosmological. The force of gravity has been extensively tested ever since Isaac Newton (1687) provided the first mathematical theory. Any discrepancy between observations and what the laws of gravity predict could signal either the necessity to revise the theory itself (as happened with Einstein's revolutionary theory of general relativity), or the discovery of an unknown and previously invisible gravitating mass. An early example of the latter occurred in 1846 when astronomers noticed persistent anomalies in the motion of Uranus, departures from the movements that were predicted by Newtonian gravity. These observations led the French astronomer Urbain le Verrier to postulate the existence of a new mass in the vicinity of Uranus, which was soon identified as a new planet: Neptune. The first evidence of the existence of dark matter (DM), an unknown source of gravity diffused throughout the universe, emerged in a similar way.
Early Evidence
Early assumptions about the significant presence of DM was based on the observation of extragalactic objects, and indeed the largest among them: galaxy clusters. Galaxy clusters are gravitationally bound structures containing hundreds of galaxies. In 1933, Fritz Zwicky, a Swiss-American astronomer, began to study the motions of individual galaxies within the Coma Cluster and noticed that the galaxies orbiting the centre of the cluster had surprisingly large velocities. Galaxies with such large velocities could only remain bound in the cluster if the gravitational field acting on them was much stronger than estimations generated from observations of visible matter. Zwicky, in order to make sense of these measurements, calculated the required gravitational potential energy in the cluster and found that its source could only be a mass an order of magnitude higher than what was visible to the telescopes. He concluded: "If this should be verified, it would lead to the surprising result that dark matter exists in much greater density than luminous matter." At first, this finding did not attract much attention and was interpreted as a problem of missing luminosity, the assumption that our observations of galaxies and stars must somehow be obscured rather than that mass itself was missing.
The effort to explore this issue further nonetheless continued, focusing on intermediate scales such as regular rotational galaxies like our own galaxy. We expect that stars in the outskirts of galaxies will have lower rotational velocities because the gravitational force rapidly declines with the distance from the centre. After inconclusive early observations, technological advances in the 1960s made it possible to perform these measurements at increasingly larger distances from the galaxy centre. Observations of Andromeda with a new spectrographic instrument pioneered by Kent Ford (and mostly performed by his student Vera Rubin) clearly demonstrated that the velocities of stars remained unchanged up to very large distances from the centre instead of decreasing as expected. This work, together with similar measurements obtained in radio observations by Morton Roberts, are typically credited with providing the final piece of evidence that convinced the community of the existence of a new source of mass invisible to telescopes but present on a range of scales from a single galaxy to galaxy clusters.
The Role of DM in the Evolution of the Universe
Cosmologists studying the evolution of the universe soon realized that if the distribution of matter started in an almost perfect state of homogeneity with only tiny fluctuations around a mean constant density, such small perturbations would, due to the attractive force of gravity, grow over cosmological time and result in the huge structures (i.e. galaxies and galaxy clusters) that we observe in the universe today (see images below).
Computer simulations were necessary to study the role of DM in such a highly non-linear process. The first such simulations were performed in the 1960s and today cover the dynamic range from cosmological evolution to galaxy formation. The simulations demonstrated that the presence of a non-relativistic and almost collisionless fluid, dubbed cold DM, is actually necessary to form the universe as we see it today. DM indeed ensures the required growth of structures throughout the history of the universe with the 'visible' matter falling into the potential wells created by collapsed DM structures named DM halos. If the matter in the universe consisted mainly of ordinary particles (interacting with each other and with photons, and thereby exerting a pressure that opposes the growth of perturbations), the gravitationally bound structures we observe today, from dwarf galaxies to galaxy clusters, could not have been formed.
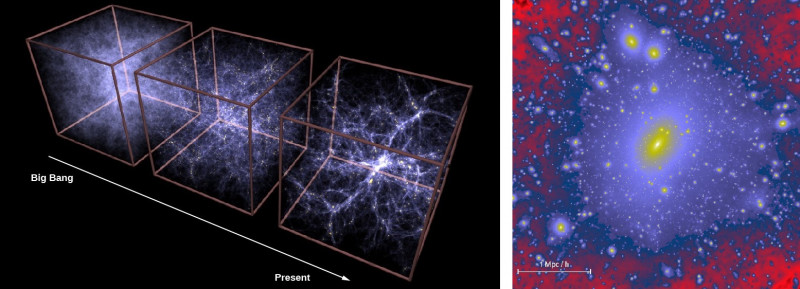
What we Know about DM
In addition to DM and the usual matter (baryons) discussed above, the current standard cosmological model contains an even more puzzling dark energy component, which could provide explanations for a wide range of observations, including the temperature of the cosmic microwave background and the large-scale distribution of galaxies beyond the size of cluster. These observations allow us to determine that the amount of DM today is approximately 25% of the total energy density of the universe, with ordinary matter making up only 5%. We also know that DM is indeed dark, that is, that it does not interact with photons, which in turn implies that it is electrically neutral. We know from N-body simulations that DM must be a non-relativistic and collisionless/pressureless fluid. Using these hypotheses, the same simulations gave us a detailed picture of DM clustering, and it has now become clear that all galaxies sit at the centres of DM halos extending far beyond the galaxies' size. DM halos themselves contain large numbers of sub-halos, smaller structures accreted over the course of time (see right image above). The DM density estimated in our solar system is such that, if DM particles were to have proton-like mass, it would be about one particle per cubic centimeter.
What is DM?
The Standard Model of particle physics contains no suitable particle to explain the full set of inferred DM properties. DM thus might offer us a glimpse of unknown physics beyond the Standard Model, and at the least provide critical evidence that our picture of the fundamental particles and their interactions is incomplete. The class of DM candidates that has attracted the most attention in recent decades are so-called weakly interacting massive particles or WIMPs. WIMPs appear to be the perfect candidate: new particles at the weak scale, that is with a mass about 100 times the mass of a proton (i.e. about 100 GeV, with GeV corresponding to a proton mass), they would naturally have been produced in the right abundance in the early universe.
However, WIMPs are not the only candidates. Many other contenders, to various degrees motivated by unrelated open problems in the Standard Model, span orders of magnitude in mass, ranging from ultra-light bosons often referred to as fuzzy DM (mass 10-22 eV) to the WIMPZillas with masses approaching the Planck scale (masses <~1018 eV). In addition, models in which DM does not consist of particles but of macroscopic objects (such as primordial black holes) also present viable candidates.
Detecting DM
Gravity is the portal that led to the discovery of DM and is still the only force known to mediate DM interactions. If the DM particle is to complete the Standard Model of fundamental particles, however, it should have small but non-zero interactions with it, opening up the possibility for detection via forces other than gravity. Intrigued by this perspective, a composite scientific community, from particles physicists to astronomers, set out to study the promising GeV-TeV range of masses with unprecedented precision. As a result, several experiments during the past decades have come online that will explore the various types of interactions between Standard Models particle and the 'dark' sector.
Particle Colliders: The proton-proton collider LHC at CERN in Geneva is a true achievement of mankind, with over 20 countries (including Slovenia) participating in the effort. It was started in 2008, and by 2012 had already produced the discovery of the Higgs boson, the last particle of the Standard Model. The hope is that, before it retires, the collider will offer a glimpse of physics beyond the Standard Model. DM particles could be produced in collisions between protons at TeV energies. If so, they would promptly escape the detector and could therefore be searched for as 'missing mass' in recorded events.
'Direct' detection: The earth effectively experiences a DM 'wind' as it moves through the DM halo of the galaxy on its orbit around the Sun and the galaxy centre. Because of this, it is possible that dark matter particles scatter against nuclei of atoms in our detectors, and this provide a direct means to search for the signals of the dark matter particles. This technology has become extremely advanced with the field dominated by ton-scale liquid gas detectors that have sensitivities several orders of magnitude higher than early technology.
Astrophysical probes: Astrophysical probes of DM aim to deduce some of its particle properties from the available astrophysical data. This kind of search is experiencing a renaissance recently, as many new, highly sensitive astrophysical experiments have been conducted, including the use of gravitational waves as an entirely new messenger. The community is actively working to share these data and analyse them in the most comprehensive ways possible. The aim is to detect the annihilation or the decay of DM particles into Standard Model particles that might be detected as cosmic rays. High-energy photons, or gamma rays, are particularly interesting cosmic rays messengers as they travel in straight lines and are abundantly detected in current experiments. Slovenia, and particularly the University of Nova Gorica, are very active in the field of gamma-ray astronomy, contributing to the largest next-generation gamma-ray experiment: The Cherenkov Telescope Array or CTA, expected to come online in 2022. One of our projects deals with the potential of the CTA to detect DM annihilation at the centre of our galaxy, possibly the brightest source of DM-induced cosmic rays in the sky. We have demonstrated that the CTA could in theory provide the final word on the WIMP DM hypothesis (see images below).
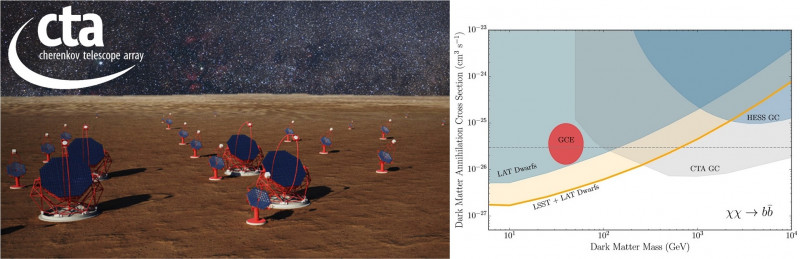
While the true nature of DM remains a mystery, the quest to discover its secrets has aroused human curiosity and ingenuity and propelled technological developments for over half a century. After the 1990s, which were dominated by the search for WIMPs, we are today in a vibrant period where many new avenues are being explored, and we are able to benefit from an abundance of data and novel analytical techniques, based for instance on machine learning. Looking into the future, DM investigations will take advantage of the observational revolution taking place in astrophysics and cosmology, and we will able to learn more than ever about its nature by continually increasing the sensitivities of a range of DM models.